Experimental digital twins
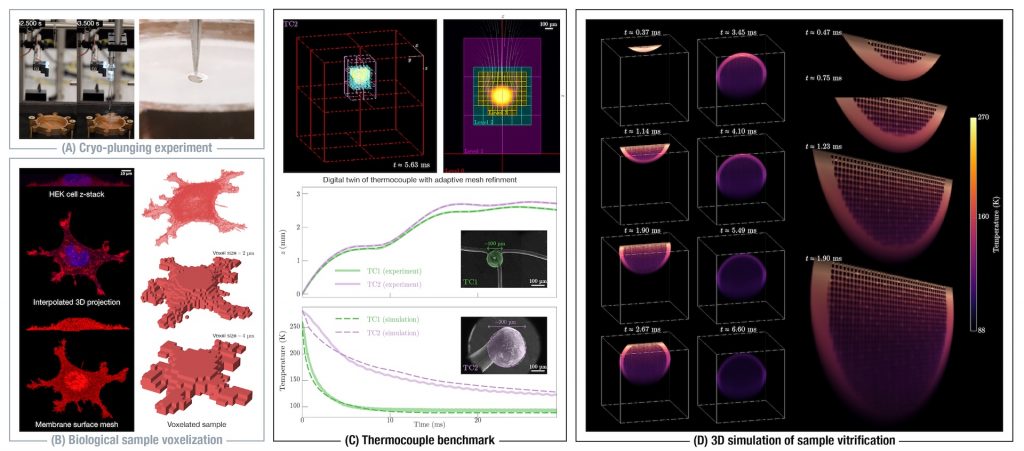
Digital twins are a powerful complement to experimental science, providing access to data that is unattainable through physical experiments alone. By simulating experimental environments on computers, this data-driven approach reveals parameters and variables that traditional experimental methods cannot measure or track, ultimately leading to theories that can aid in the development and design of experiments.
In collaboration with Profs. Max Prigozhin (Harvard) and Chris Rycroft, I developed 3D simulations of plunge-freezing for sample vitrification in cryogenic electron microscopy (cryo-EM). In this process, known as cryo-plunging, biological samples are plunged into cryogen (≈ 90 K). Cryo-plunging is critical for achieving high-quality images, but cryo-EM practitioners report success rates below 25% on initial attempts. Image quality is tied to cooling rates, but measuring them in samples smaller than 50 μm is nearly impossible, leaving much of this rapid cooling process unknown. Establishing a robust theoretical framework is crucial for guiding experimentalists, yet it remains inherently challenging for complex nonlinear systems. Therefore, simulation becomes an indispensable tool for understanding and improving the cryo-plunging protocol, optimizing it for larger samples, and ultimately saving time and resources. A major modeling challenge is to capture the extreme scale difference between the EM grid (≈ 3 mm) and the sample (≈ 10 μm), as well as the extreme temperature gradient between the sample and the cryogen—with differences of over 100 K and cooling rates exceeding 105 K/s.
I developed cryoflo, a digital twin of the cryo-plunging process, to systematically study the cooling process. Built upon AMReX, a parallel supercomputing code with adaptive mesh refinement capabilities, cryoflo enables efficient large-scale simulations. Using it, I digitally recreated thermocouple experiments by specifying thermal and material properties, geometries, and plunging speeds. Simulated temperatures closely matched experimental data, with an average error of about 5%, benchmarking the accuracy of our simulations. Additionally, cryoflo can load actual sample geometries, prescribe plunging protocols, and visualize heat transfer and fluid motion. It also obtains temperature profiles for individual samples, revealing how they spatiotemporally vitrify. By deriving theoretical limits for vitrification time, we establish a foundation for understanding the process, confirming that our plunging experiments are improving the cooling rate. This combination of theory and simulation allows us to design new cryo-plunging protocols by exploring parameters such as sample seeding, plunger geometry, and speed. This digital twin can significantly optimize sample preparation, enabling more efficient freezing protocols and making it possible to plunge-freeze thicker samples—once considered unfeasible. This work is currently in preparation for a numerics-focused paper, along with a collaborative publication.
I am also part of an inter-university collaboration on redox flow batteries (RFBs), led by Prof. Mike Aziz (Harvard). RFBs offer a scalable energy storage solution to the energy crisis, but face inefficiencies due to heterogeneous distribution of reactive species across electrodes. Our project combines experimental work on 3D-printed electrodes with AMReX-based simulations. Building on my experience with cryoflo, I am currently developing large-scale simulations to analyze flow profiles and state-of-charge, optimizing electrode designs to improve battery performance.